Introduction
The development of Ecosystem-Based Fisheries Management (EBFM) requires both an integrated understanding of ecosystem structure and function and an explicit consideration of multiple management objectives (Larkin, 1996; NMFS, 1999; Jennings et al., 2001; Link, 2002; Garcia et al., 2003). Further, the effects of stock-specific harvesting patterns on food web architecture require consideration of tradeoffs in management of interacting species. Here we describe patterns of energy flow and utilization for Georges Bank, a highly productive marine system located off the New England coast (Fig. 1) in support of the development of EBFM in this region. This effort is part of a broader initiative, the Energy Modeling and Analysis eXercise (EMAX), to describe ecosystem characteristics of major ecoregions of the Northeast Continental Shelf of the United States (Link et al., 2006).
Georges Bank has supported important commercial fisheries since the 16th century (German, 1987). The topographic and hydrographic features of the Bank, dominated by strong tidal mixing forces, result in high primary productivity which in turn has fueled historically high levels of fishery production. The strong rotary tidal forces on the Bank, coupled with topographic rectification results in the establishment of an anticyclonic gyre on the Bank, particularly during the stratified period. This semi-closed gyre results in the retention of planktonic organisms on the Bank and warrants its consideration as a distinct ecoregion (Fogarty and Murawski, 1998).
Recognition of the unique characteristics of Georges Bank has led to longstanding efforts to understand the ecological determinants of its high productivity. Riley (1941) explored nutrient dynamics and primary production on the Bank. Building on this development, Clarke (1946) constructed the first characterization of energy flow to the higher trophic levels for this system in a simplified food web including diatoms, mesozooplankton, benthos, and fish (Fig. 2A). Cohen et al. (1982) expanded this structure to include explicit consideration of the role of the microbial food web and nutrient recycling in energy flow in the system (Fig. 2B). Sissenwine et al. (1984) refined these estimates to highlight the importance of the production of larval and juvenile fish in this system. Here, we complement these contributions by examining a greatly expanded representation of the system structure (Fig. 3). We examine the implications of observed changes in ecosystem composition for fishery production and place our results in historical context with reference to earlier energy budgets developed for this system.
Historical Background
The history of fishing on Georges Bank can be characterized as one of sequential depletion of fishery resources and large-scale changes in the relative abundance of different ecosystem components (Fogarty and Murawski, 1998). These changes include the depletion of marine mammal populations in the 18th century (Waring et al., 2004; Clapham and Link, 2006), collapse of major fisheries such as that for Atlantic Halibut by the mid-19th century, and a series of fishery declines initiated by the arrival of distant water fleets on Georges Bank in 1961. The rapid escalation in fishing effort by these fleets resulted in an initial increase in landings as groundfish were targeted (Clark and Brown, 1977; Serchuk et al., 1994; Murawski et al., 1997; Fogarty and Murawski, 1998; Link and Brodziak, 2002). Groundfish stocks declined under heavy exploitation and the sequence was repeated for small pelagic fish (principally Atlantic herring and mackerel) and ‘other’ fish stocks (including elasmobranchs, large pelagics) throughout the 1970s (Fogarty and Murawski, 1998; Overholtz, 2002). Following the implementation of extended national jurisdiction in 1977, fisheries for invertebrate resources, notably Atlantic sea scallops, dominated the landings and value of the catch.
The fisheries on Georges Bank were effectively unregulated prior to the 1950s. With the advent of fishing activities by the distant water fleet and subsequent decimation of fishery resources, the need for direct controls on fishing was evident. The establishment of the 'two-tier' quota management system in 1974 by the International Commission for Northwest Atlantic Fisheries (ICNAF; Murawski et al., 1997) provided the nucleus for recovery of depleted stocks. This approach included explicit recognition and allowance for bycatch, discarding practices, and inter-specific interactions (Brown et al., 1976). Quota-based management was maintained under the early years of extended jurisdiction but was replaced by more qualitative measures (constraints on mesh size, legal size limits for fish, and short-term areal and seasonal closures) in 1982. When these measures failed to adequately protect fishery resource, more restrictive measures (including the use of large-scale year-round closures and limits to days-at-sea) were added in 1994 (Murawski et al., 1997; Fogarty and Murawski, 1998).
The direct and indirect effects of fishing and regulatory actions are reflected in fishery-independent abundance estimates for major fish groups (Fig. 4). As gadoid and flatfish populations declined under heavy exploitation, elasmobranch (skates and spiny dogfish) populations initially increased. However, a redirection of fishing effort on these species as groundfish continued to decline resulted in reduced elasmobranch populations. Sharp reductions in fishing pressure resulted in increases in herring and mackerel populations through the 1990s (Fig. 4; Overholtz, 2002). The time period covered in our analysis (1996–2000) therefore encompasses a period of high pelagic fish abundance, the start of recovery of some groundfish populations, and lower elasmobranch abundance relative to the preceding decade.
Methods
Modeling Approach
We constructed mass balance ecosystem representations of Georges Bank using two well established models, Ecopath (Christensen and Pauly, 1992; Walters et al., 1997) and Econetwrk (Ulanowicz and Kay, 1991; Ulanowicz, 2004) The properties of these models have been noted elsewhere (Heymans and Baird, 2000; Allesina and Bondavalli, 2003; Kavanagh et al., 2004; Ulanowicz, 2004; Dames and Christian, 2006).
The energy balance model for each compartment in both Ecopath and Econetwrk can be expressed as:
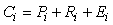
where Ci is consumption, Pi is production, Ri is respiration, and Ei is egestion (unassimilated food) of the ith compartment.
In Econetwrk, the production of any given compartment represents a gross estimate rather than net production (as such, we do not make direct comparisons of those estimates here to prior energy budget estimates). In Ecopath, the production for a closed system is given by:

where Yi represents fishery removals (yield), Bi is biomass, M2i is predation mortality and MOi is all other sources of mortality in the ith compartment. The model can be readily extended to include net import or export terms from each compartment and accumulation of biomass at each node. Other mortality (MOi) can be expressed as:
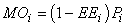
where EEi is the ‘ecotrophic efficiency’ (0 < EEi < 1).
The mass balance model can then be expressed:

where Bi is the Biomass in compartment i, (P/B)i is the production to biomass ratio, EEi is the ‘ecotrophic efficiency’ (fraction of total production that is utilized in the system); Yi is the catch for compartment i ; Bj is the biomass and (C/B)j is the consumption to biomass ratio for predator j; and DCij is the diet composition of predator j (fraction of biomass comprising prey i in the diet of predator. In the following, we have assumed no net migration during the time period considered.
Balancing protocols
Following the specification of the input variables, we made an initial calculation of the difference between the inflows and outflows from each compartment. For compartments exhibiting large discrepancies, we re-examined the input information for consistency and made comparisons with similar inputs from other systems, making adjustments as necessary. We then employed balancing options in both software packages to provide estimates of the steady-state flows. Ecopath employs a statistical balancing procedure to constrain the estimates for the underdetermined system of equations. Ecopath allows the specification of an index of reliability (or ‘pedigree’) for each element to provide weighting options for estimation. Econetwrk employs a ‘manual’ balancing procedure in which an extended matrix comprising elements for the exchanges among compartments, respiration, imports, and exports is adjusted using successive transformations (Allesina and Bondavalli, 2003). Econetwrk allows for ‘locking’ estimates thought to be well determined and therefore provides an implicit weighting procedure. Following each balancing procedure we re-evaluated inputs showing large deviations from mass balance or unrealistic estimates for certain diagnostic measures such as the ratio of respiration to consumption in Econetwrk and the ecotrophic efficiency in Ecopath. Adjustments were made to the inputs to obtain more realistic estimates and the balancing protocols repeated until stable estimates were obtained.
The initial estimates for inputs used in developing the mass-balance models are provided in Table 1. The initial proportional diet compositions used in balancing are given in Table 2, which, when coupled with consumption:biomass (C:B) estimates produce flows among compartments.
Ecopath provided a range of options for autobalancing and we employed both minimizing the sum of excess Ecotrophic Efficiency (EE) and minimizing the maximum current EE. Additionally, we used the Ecopath pedigree table to set confidence values for biomass, production:biomass (P:B), C:B, diet and catch for autobalancing. We relied on the pedigree approach since it provided a way to weight the data sources with their relative degree of confidence. Large-scale changes were necessary to gelatinous zooplankton, squid, demersal omnivores, macrobenthos-other, and shrimp. We reduced the C:B ratio, diet composition, and biomass (B) for gelantinous zooplankton, C:B and P:B of demersal ominivores, C:B of macrobenthos mollusks, B and C:B of macrobenthos other, diet composition of predators of small pelagics, P:B and B of shrimp.
Econetwrk used the DATBAL routine to balance the network, mainly using consumption:biomass and respiration:biomass (R:B) as the tuning parameters. For Econetwrk, significant changes to gelatinous zooplankton (B, C:B), macrobenthos other (B, R:B, C:B), larval fish (B), and shrimp (B, C:B, R:B) were also initially required to achieve network balance. We further found it necessary to modify megabenthos filterers, macrobenthos mollusks, medium pelagics, demersal omnivores, small pelagics anadromous, and small copepods to achieve a balanced network.
Data Sources
We developed an ecosystem network model for Georges Bank comprising 33 nodes based on ecosystem monitoring programs of the Northeast Fisheries Science Center (NEFSC) and, where necessary, estimates derived from the literature for similar ecosystem components (Fig. 3). The two fisheries nodes were not modeled directly; we also dealt with dissolved organic carbon by proxy using particulate organic detritus; thus that node was also not directly modeled. Unless otherwise noted, the estimates are for the period 1996–2000. A full description of data sources and analytical methods used to derive estimates of biomass, production, consumption and respiration is provided by Link et al. (2006; http://www.nefsc.noaa.gov/nefsc/publications/crd/crd0615).
Here, we briefly recapitulate the main elements of the model parameterization for the major functional groups represented in the network model. These nodes represent the full range of the biological hierarchy, with organisms spanning <10 µm to >30 m. Most network nodes represent a broad range of functionally similar taxa, but also integrate across a wide range of diversity and ecological functionality. The species in each node can be found in a more detailed set of documentation (Link et al., 2006). We particularly chose to include elements of the microbial loop (Azam et al., 1983) to help in the balancing, to better reflect reality, and to acknowledge some of the fundamental shifts in views of how the oceans function. Again, our emphasis was on small pelagics so some of the nodes that may not greatly interact with those species were not necessarily grouped as they might have been with a different focus.
Phytoplankton and Primary Production
We derived estimates of phytoplankton biomass (mg Chl a m-2) in the water column using in situ vertically integrated profiles of chlorophyll a pigment (O’Reilly and Zetlin, 1998) and remotely sensed estimates of near surface Chl a coupled with the vertical profile model of Morel and Berthon (1989). We estimated primary production using an adaptation of the method of Behrenfeld and Falkowski (1997) based on remotely sensed surface chlorophyll concentration, photosynthetically active radiation (PAR) and sea surface temperature (SST).
Bacterioplankton
Direct estimates of biomass and production of bacterioplankton on Georges Bank have not been routinely made. In our analysis, we assumed that bacterial secondary production is approximately 10% of primary production. Bacterial consumption was taken to be 40% of the net production.
Microzooplankton
Direct observations of microzooplankton biomass on Georges Bank have not been routinely made. We employed an estimate of microzooplankton : phytoplankton biomass ratio for this quantity. There is a wide range of literature values for the ratio of microzooplankton : phytoplankton biomass ranging from 11–99% (Baretta-Bekker et al., 1994; Pomeroy, 2001). We used a ratio of 0.13 for Georges Bank which is similar to values for unfertilized North Sea mesocosms (Baretta-Bekker et al., 1994) and Narragansett Bay (Monaco and Ulanowicz, 1997).
Copepods
The mean abundance of copepods was calculated for bimonthly subsets of standard NEFSC Ecosystem Monitoring (ECOMON; a synoptic plankton survey for the region). The copepods were partitioned in ‘large’ and ‘small’ categories. Copepod abundance was converted to biomass using the length to wet weight equations given by Pearre (1980). Zooplankton production was calculated according to Huntley and Lopez (1992) accounting for zooplankton biomass and mean integrated water column temperatures down to maximum sampling depths. Daily consumption for both copepod size groupings was taken to be 0.20 of biomass based on a range of species and life histories stages.
Gelatinous Zooplankton
Gelatinous zooplankton biomass was estimated from NEFSC ECOMON cruises. To convert from length to dry weight, we used the conversion relationship (Reeve and Walter, 1976) derived originally for ctenophores. Dry weight was then taken to be 4.48% of wet weight (Pages, 1997). To estimate production, we employed a production to biomass ratio of 40. Consumption was estimated under an assumed clearance rate of approximately 40% body weight per day.
Micronekton
The micronekton group (including euphasiids, mysids, hyperiid amphipods, chaetognaths and pelagic gammarids) was estimated using information from NEFSC ECOMON cruises. Mean abundance was converted to dry weight for from Avery et al. (1996) for gammarids and Sameoto (1971) for chaetognaths. Growth was estimated at 0.04 d-1 and consumption at 0.10 d-1 based on an assumption growth is similar to that of juvenile cod of approximately the same size (Peck et al., 2003).
Macrobenthos
For the purposes of this analysis, the macrobenthos group included epibenthic and infaunal crustaceans, polychaetes, mollusks, nemerteans, echinoderms, coelenterates, and tunicates. Large-bodied crustaceans and echinoderms are included under the megabenthos category (see below). Benthic biomass data were not available for the period 1996–2000. Accordingly, we used previous benthic grab survey data for this region. (Steimle, 1990; Reid et al., 1991; Theroux and Wigley, 1998). To estimate production for each of the macrobenthic taxa, we employed the P:B ratios of Steimle (1985, 1987). Consumption rates were estimated using a C:B ratio of 0.10.
Megabenthos
Fisheries independent survey data from the NEFSC Bottom Trawl Survey were used to estimate biomass density for large arthropods. Sea star biomass was derived from comprehensive regional benthic grab data (Theroux and Wigley, 1998). Bivalve biomass was estimated from NEFSC clam and scallop surveys. Production for bivalves was estimated using a P:B ratio of <10 based upon a range noted in Dame (1996). Consumption was estimated using a C:B ratio of 10 (Dame, 1996). For non-bivalves, the P:B ratio of 1.5 was used based on the assumption that as large, active invertebrates, P:B should resemble that of squid and shrimp. Consumption for non-bivalves was estimated using a C:B ratio of 13.5 based again on the assumption that shrimp should resemble other large benthic invertebrates.
Shrimp
We employed an estimate of shrimp biomass based on limited beam trawl sampling in the southern New England-Mid-Atlantic region for this purpose. Production was estimated using a P:B ratio of 1.5 and consumption was estimated using a C:B ratio of 13.5 based on ratios derived for similar species.
Larval fish
Larval fish biomass was derived from 1977–1987 NEFSC ichthyoplankton sampling. Dry weight was estimated for each 1 mm abundance group averaged over all seasons of sampling using the length to weight relationship for Atlantic cod which was representative of that for other species encompassing a range of larval body shapes (Laurence, 1979). Growth and consumption rates for larvae are based on laboratory studies at the NMFS Narragansett laboratory and direct field observations (Buckley and Lough, 1987). We used these values to scale the initial inputs for production and consumption of larval fish, assuming that the species studied in vivo were generally representative (in terms of magnitude) of these rates.
Juvenile and Adult Fish
We derived estimates of the biomass of juvenile and adult fish in three major categories (small pelagic, medium pelagic, and demersal fishes) based on NEFSC bottom trawl surveys in spring and autumn. The mean catch per tow was adjusted for the area swept by the net and for catch efficiencies for each taxonomic group. Determination of relative catch efficiencies differed by taxonomic group and used either literature values for these species (Edwards, 1968; Harley et al., MS 2001) or newly derived estimates described in Link et al. (2006). Where available, age-structured information was used to estimate growth rates which were then used to determine production rates for individual species. We then estimated production to biomass ratios for these species and applied the ratios to species within the same taxonomic category for which full age-structured information was not available. Consumption estimates were based on information from mean stomach content weight and diet composition from the NEFSC food habits database. These estimates utilized the Eggers (1977) equation for consumption.
Large Pelagics
For tunas we used estimates of biomass and production derived from International Commission for the Conservation of Atlantic Tuna (ICCAT) stock assessment information where available (bluefin and bigeye tunas). For other tuna and billfish species we used information on the ratio of the catch of these species to bluefin catches in Japanese longline data (Hoey et al., 2002) and scaled these estimates relative to the bluefin biomass. P:B ratios derived for bluefin and bigeye were applied to other tuna species literature values were applied to billfish as described in Link et al. (2006). Consumption estimates for bluefin tuna were applied to other tunas and assumed values were applied to billfish species.
To estimate blue shark abundance, we used the ratio between blue shark and bluefin tuna catch rates from Hoey et al. (2002) to produce a raising factor to scale blue shark numbers during 1996–2000 to bluefin tuna numbers for the same period. For all other sharks, the ratio of their catch rates to blue shark catches were used to determine biomass. Production for all sharks was estimated by assuming a P:B ratio of 0.1.
Consumption by sharks in the four regions was estimated from daily ration estimates for blue and mako sharks (Stillwell and Kohler, 1982). An average of these two values was used to estimate consumption for the other shark species.
Baleen Whales and Odontocetes
We determined biomass of baleen whales and odontocetes from NEFSC shipboard and aerial sighting surveys conducted during 1998 (Palka, 2005). Production estimates were derived assuming a maximum population growth rate of 4%. Estimates of consumption rates were derived using estimates of daily feeding rates. The feeding rate per individual per day is defined as a percentage of its biomass. There is an inverse relation between feeding rate and body weight (Sergeant, 1969).
Seabirds
We employed estimates of seabird abundance for the period 1978–1980 (Powers, 1983). Abundance estimates were converted to biomass using average species specific weight from Powers and Bachus (1987). Consumption by seabirds was estimated from daily ration estimates from an energetics equation (Innes et al., 1987).
Fishery Removals
Data on U.S. and Canadian landings (live weight in metric tons) of demersal and pelagic managed species from the years 1996–2000 were obtained from stock assessment reports compiled by NEFSC. For stock units including but not limited to Georges Bank, allocation of the catches to Georges Bank was made using catches by statistical area and expert opinion of the stock assessment scientists. Commercial landings of large pelagic species were obtained from ICCAT reports. These data were apportioned by using distribution maps and making assumptions about the percentage landed on the continental shelf.
Where available, commercial discard estimates were obtained from stock assessment reports. Discards for all the other groups were estimated as percentages of the landings; for example discards for the demersal species were assumed to be 30% of landings during 1996–2000 (Alverson, 1997). Discard estimates for megabenthos were assumed to be 0.0001 of biomass, while gelatinous zooplankton, mesopelagics, and larval fish were assumed to be 0.000001 of biomass, and finally seabirds were 0.01 of biomass.
Comparisons with Prior Energy Budgets
We examined information on Georges Bank resulting from studies conducted in two prior time periods. In general terms, we present a set of common network metrics to facilitate comparisons across all eras. The 1940s results on Georges Bank come from Clarke (1946; Fig. 2A). The 1980s results come from Cohen et al. (1982) and Sissenwine et al. (1984; Fig. 2B).
We present biomass, production, and vital rate information from these different studies compared to the EMAX results. These were trophically aggregated into four simple nodes: primary producers, zooplankton, benthos, and fishes, which, particularly in the latter years, have been highly integrated across ecosystem compartments. We present the total number of flows from each era and the consumption estimates of zooplankton and fishes as a specific example of these flows. We also present the modal number of trophic levels and estimated fishery yields from each era. The units from each study were converted to kcal m-2 yr-1 for rates, or kcal m-2 for standing stocks, to facilitate comparisons.
Results and Discussion
Balanced Model Results
Estimates of mean trophic level, ecotrophic efficiency, and selected network metrics for each model compartment are provided in Table 3. For simplicity we only present results from Ecopath in Table 3. The estimate of the proportion of biomass utilized from each compartment is reflected in the ecotrophic efficiency parameter. The ecotrophic efficiency for phytoplankton was 0.674, reflecting a substantial fraction (i.e., >30%) of primary production not fully utilized by other components of the ecosystem. The ecotrophic efficiency was also notably low for most components of the zooplankton (particularly for micronekton). These values suggest that lower trophic levels in this ecosystem are either 1) not fully utilized by their consumers, 2) some migration of this biomass out of the model domain occurs, 3) are not fully available to upper trophic levels, 4) are being used to help balance other aspects of the system through flows to detritus, or 5) some combination of the above.
Consideration of throughput (total flows) from each ecosystem node highlights the importance of the lower trophic levels in system structure (Table 3). Again, we present results from Ecopath to characterize these system metrics. Collectively, the highest levels of throughput are attributable to the microbial food web (bacteria and microzooplankton), indicating the importance of recycling in overall system dynamics. This result is consistent with previous observations on the importance of the microbial food web and recycling on Georges Bank (Cohen et al., 1982). Phytoplankton and copepods also comprise dominant components of overall system throughput. Benthic organisms contribute significantly to throughput. Within the fish component, throughput of larval fish and commercially exploited small pelagic fishes (particularly herring and mackerel) is particularly important (Table 3). Sissenwine et al. (1984) first noted the critical role of larval and juvenile fish in energy flow on Georges Bank. The importance of herring and mackerel in throughput reflects the current dominance of these species in the total fish biomass of the system.
Ascendency is a measure of the size, structure, flows, and organization (information content) of the flows. Higher levels of ascendancy reflect higher levels of specialization and efficiency in the food web. Developmental capacity is the upper limit of ascendancy for the food web. For our system, ascendancy is 21% of capacity (Table 3) and the estimate of overhead (the difference between developmental capacity and ascendancy) is relatively high. These observations are consistent with a system with relatively high resilience to perturbation with respect to energy flows.
To further explore structural features of the Georges Bank ecosystem, we examined patterns of direct and indirect effects exerted by each compartment on all other ecosystem nodes and indicated by the mixed trophic impact analysis. The mixed trophic impact index is expressed in relative terms that are directly comparable among compartments in the model. This analysis can provide insights into the possible implications of perturbations to the system. Here we only emphasize the stronger impacts. Positive impacts of phytoplankton are evident throughout the system (Table 4). As expected, the strongest such impacts are on ecosystem components that graze directly on phytoplankton, including copepods and filter feeding bivalves. Apparent second-order effects are evident in the positive impacts on pelagic fishes which feed on zooplankton and for baleen whales, which also prey on zooplankton. Utilization of detritus by bacteria results in a negative impact while microzooplankton exert a negative impact on bacteria (Table 4). Large copepods have a strong negative impact on gelatinous zooplankton and a strong positive effect on small pelagic fishes and baleen whales. Other macrobenthic species (small crustaceans, polychaetes, and mollusks) exert a negative impact on several benthic compartments. Commercially important small pelagic fishes have a positive impact on their predators including medium-sized pelagic fishes and sharks while other small pelagic fishes positively impact highly migratory species. Demersal piscivores exert a negative impact on several important prey groups (principally different small pelagic fish categories). Sharks show a negative effect on baleen whales and seabirds. Detritus has a strong positive impact on a number of lower trophic level compartments. Fisheries removals (landings) have a high negative effect on several groups of overexploited demersal fishes, sharks, and highly migratory species. Landings show an unexpected positive impact on baleen whales and sea birds.
Comparison with Previous Energy Budgets
The first obvious difference across the different network analyses is the increasing complexity over time. Table 5 provides some simple network macro-descriptors showing an increase in these factors over time, but the depictions of each network (Figs. 2 and 3) clearly demonstrate increasing complexity over time.
Similar levels are seen in estimates of primary production and biomass from the different eras (Fig. 5). Most values of production range between 3 500 and 4 500 kcal m-2 yr-1. Where estimates of standing stock biomass are available, they are on the order of 20–25 kcal m-2 (Fig. 5B). Previous energy budgets derived estimates of primary production on Georges Bank using the oxygen depletion method (Clarke, 1946; Fig. 2A) and 14C observations (Fig. 2B; Cohen et al., 1982; Sissenwine et al., 1984). Sissenwine et al. (1984) subsequently revised the initial 14C estimates of Cohen et al. (1982) downward (Fig. 5A). The primary production estimates using the satellite-derived estimates in the present analysis are similar to those of Sissenwine et al. (1984). We attribute the somewhat lower estimate provided by Clarke (1946; based on Riley (1941)) to important methodological differences rather than lower levels of primary production during the earlier period. Further, Clarke (1946) considered only diatom production on the Bank and apparently viewed the Georges Bank system as a classical grazing food chain linking diatoms to mesozooplankton. In contrast, subsequent energy budgets recognized the importance of the microbial food web and recycling in the production dynamics of the Bank (Cohen et al., 1982).
These relatively similar production estimates also confirm that by all standards, this has remained a highly productive system relative to many other places in the World’s oceans. Georges Bank is and has been one of the most productive marine ecosystems on the planet (Grosslein et al., 1980; Cohen et al., 1982; Sissenwine et al., 1984; O’Reilly et al., 1987; Bax, 1991), an observation reinforced by this study.
Estimates of production and biomass are similar for most other biotic groups across time, except zooplankton production (Fig. 6A, B). This is due to the much higher production rates used (Figs. 6A and 7) for zooplankton in the contemporary network analyses. Zooplankton production estimates differed very substantially among the studies considered here for a couple of reasons. The estimates of Cohen et al. (1982) and Sissenwine et al. (1984) were based on a P:B ratio of 7 borrowed from estimates for the North Sea. Estimates derived from the Huntley-Lopez formulation employed in the present analysis are markedly higher (Figs. 6A and 7). The much lower estimates developed by Clarke (1946) reflect limited sampling and the use of coarse-mesh plankton nets to characterize the mesozooplankton community. The change in zooplankton production estimates from the Cohen-Sissenwine energy budgets to those employed in EMAX provides an important perspective on production dynamics on the Bank. Cohen et al. (1982) and Sissenwine et al. (1984) considered their low estimates of the ratio of secondary to primary production to be due to export of zooplankton from the Bank. Although net loss of zooplankton is clearly possible (cf. ecotrophic efficiency above), our estimates suggest a much higher ratio of secondary to primary production in line with estimates from other systems. This reflects the recognition that there is likely more primary production available to zooplankton on Georges Bank due to nutrient recycling, earlier studies likely under-estimated zooplankton production, and the improvements resulting from incorporating the recent available data on zooplankton production rates.
Other vital rates changed over time as well (Fig. 7). Most of these changes are amalgamated in the entire network and do not exhibit major changes in production or biomass of most biotic groups.
Estimates for fisheries yields are mostly similar across the different eras of Georges Bank network studies (Fig. 8). This is despite the fact that energy budgets for the different time periods reflect markedly different species compositions for exploited fish populations (Fig. 4). Standardized estimates of fish biomass were not available for the period covered by the Clarke (1946) analysis from either fishery-independent or fishery-dependent sources. It can be inferred, however, that fish biomass was higher than those experienced during the period of impact by the distant water fleets; it may well follow that the Clarke estimates of fish production were underestimates due to limited data available to him at the time. The Cohen et al. (1982) and Sissenwine et al. (1984) studies provided estimates of fish production for two time periods: 1964–1966 and 1973–1975. The former represented a period of declining groundfish abundance. By 1973–1975, haddock and other groundfish populations had effectively collapsed as had the herring and mackerel fisheries (Fig. 4). The estimates of fishery yield from the Cohen-Sissenwine study actually reflect estimated rather than realized values of maximum sustainable yields subject to different management constraints. Thus they are higher than the values resulting in the contemporary EMAX or 1940s network analysis. Estimates of fish biomass by Sissenwine et al. (1984) were similar to those in the EMAX study despite lower estimates of production (Fig. 7B).
Besides the Sissenwine et al. (1984) study, these estimates of fishery yield on Georges Bank range between 2–5 kcal m-2 yr-1(Fig. 8). Converting to biomass and accounting for the area of Georges Bank translates into roughly 90–220 thousand metric tons per year. The overall consistency (within half an order of magnitude) over time of these yield estimates is quite remarkable considering all the potential sources of variability and changes in how we have estimated these values. Yet again these observations confirm that this is a highly productive ecosystem compared to most other marine food webs (Bax, 1991).
Because of our focus on fishery-related issues, our network model incorporates a greatly expanded representation of fish functional groups relative to previous energy budgets (Table 5). The Sissenwine et al. (1984) update of Cohen et al. (1982) had two functional groups, demersal and pelagic fishes along with a larval/juvenile fish compartment while ours specified ten groups (including one for squid in the small pelagics category). Our analysis encompasses a more detailed representation of fishery removals by specifically accounting for discarded catch. Additionally, previous energy budgets for this system have not included marine mammals and sea birds. We specify two functional groups of marine mammals and one for seabirds. Although the biomass and production levels of these groups are low, concerns over fishery-related impacts on their populations warrants special consideration in defining the structure of the Georges Bank ecosystem.
Conclusions
Georges Bank is a highly productive continental shelf system. Our analysis indicates a notable influence of primary producers throughout the system, indicative of strong bottom-up forcing. Factors that alter levels of primary production therefore can be expected to exert widespread effects on system dynamics. Climate-related changes in stratification or other factors may ultimately prove very important in nutrient dynamics and primary production processes on the Bank.
The properties of the Georges Bank food web have certainly responded to environmental and human influences, yet not to the point that the major, systemic features of the network are fundamentally different over the past half of a century or more. Several key properties of Georges Bank, and we suspect many natural systems, remain conservative properties (within bounds of variation). This is likely to be especially true when viewed from a more aggregate or systemic perspective.
The microbial foodweb is shown to be extremely important in the energy flow of the system, indicated by high levels of throughput for these components. The recognition of the importance of recycling on the Bank does hold important implications for the amount of energy that can be transferred to higher trophic levels since the pathways are more complex than those of a strictly diatom-based food web (Townsend and Pettigrew, 1997).
We estimate secondary production to be substantially higher than previous analyses of the Georges Bank system, providing a different perspective on what had been perceived as a lower than expected ratio of secondary to primary production and attributed to export processes. A relatively high level of under-utilized zooplankton production on the Bank, indicated by the estimates of ecotrophic efficiency, may nonetheless suggest the possible importance of transport and advective processes.
Relative species composition of the fish community differed markedly in our analysis relative to previous energy budgets for this system, with a dominance by small pelagic fishes. Despite these differences, the estimated biomass levels for most aggregate groups were roughly comparable to prior energy budgets.
The EMAX network analyses demonstrated that small pelagic fishes are important for overall energy flow in the system. Similar to the findings of the Cohen-Sissenwine study for pre-recruits, these mid trophic level forage fish serve as a critical set of links between upper trophic level biota and lower level producers.
Finally, estimates of system developmental capacity and overhead suggest a highly resilient system. The high degree of throughput, the highly inter-connectedness of all the biota in this ecosystem, and history of comparable biomass in major functional groups across 60 years of the different studies suggest that if one biomass flow pathway is altered, another pathway compensates such that overall changes in standing stock biomass at a given trophic level are minimized. These conditions are all indicative of a highly productive and highly resilient system.
Acknowledgements
We express our appreciation to our colleagues at the Northeast Fisheries Science Center who have contributed to the longstanding ecosystem monitoring programs that provide the basis for much of this analysis. We are also grateful to Edward Cohen (deceased), Marvin Grosslein, and Michael Sissenwine for their prior efforts on Georges Bank energy budgets. We are grateful to Wendy Gabriel, Fred Serchuck, and Thomas Noji for their critiques of an earlier version of this manuscript. We thank the NESFC executive staff who allowed all of us to dedicate a portion of our time to the EMAX.
References
ALLESINA, S., and C. BONDAVALLI. 2003. Steady state of ecosystem flow networks: a comparison between balancing procedures. Ecol. Modelling, 165: 221–229. doi:10.1016/S0304-3800(03)00075-9
ALVERSON, D. L. 1997. Global assessment of fisheries bycatch and discards: a summary overview. In: Global trends: fisheries management. E. K. Pikitch, D. D. Huppert and M. P. Sissenwine (eds.). Am. Fish. Soc. Symp., 20: 115–125.
AVERY, D., J. GREEN, and E. DURBIN. 1996. Distribution and abundance of pelagic gammarid amphipods in Georges Bank and Nantucket Shoals. Deep Sea Res. II., 43: 1521–1532. doi:10.1016/S0967-0645(96)00045-8
AZAM, F., T. FENCHEL, J. G. FIELD, J. S. GRAY, L. A. MEYER-REIL, and F. THINGSTAD. 1983. The ecological role of water-column microbes in the sea. Mar. Ecol. Prog. Ser., 10: 257–263. doi:10.3354/meps010257
BAX, N. J. 1991. A comparison of the fish biomass flow to fish, fisheries, and mammals on six marine ecosystems. ICES Mar. Sci. Symp., 193: 217–224.
BARETTA-BEKKER, J. G., B. REIMANN, J. BARETTA, and E. K. RASMUSSEN. 1994. Testing the microbial loop concept by comparing mesocosm data with results from a dynamical simulation model. Mar. Ecol. Prog. Ser., 106: 187–198. doi:10.3354/meps106187
BEHRENFELD, M. J., and P. G. FALKOWSKI. 1997. Photosynthetic rates derived from satellite-based chlorophyll concentration. Limnol. Oceanogr., 42: 1–20.
BROWN, B. E., J. A. BRENNAN, M. D. GROSSLEIN, E. G. HEYERDAHL, and R. C. HENNEMUTH. 1976. The effect of fishing on the marine finfish biomass in the Northwest Altantic from the Gulf of Maine to Cape Hatteras. ICNAF Res. Bull., 12: 49–68.
BUCKLEY, L. J., and R. G. LOUGH. 1987. Recent growth, biochemincal composition, and prey field of larval haddock (Melanogrammus aeglifinus) and Atlantic cod (Gadus morhua) on Georges Bank. Can. J. Fish Aquat. Sci., 44: 14–25.
CHRISTENSEN, V., and D. PAULY. 1992. On steady-state modeling of ecosystems. In: Trophic models of aquatic ecosystems. V. Christensen and D. Pauly (eds.). ICLARM, Manilla. ICLARM Conf. Proc., 26: 14–19.
CLAPHAM, P. J., and J. S. LINK. 2006. Whales, whaling and ecosystems in the North Atlantic Ocean. In: Whales, whaling and ecosystems. J. A. Estes, D. P. DeMaster, D. F. Doak, T. M. Williams and R. L. Brownell (eds.). University of Chicago Press. p. 314–323.
CLARK, S. H., and B. E. BROWN. 1977. Changes in biomass of finfishes and squids from the Gulf of Maine to Cape Hatteras, 1963–74, as determined from research vessel survey data. Fish. Bull., 75: 1–21.
CLARKE, G. L. 1946. Dynamics of production in a marine area. Ecol. Monogr., 16: 321–337. doi:10.2307/1961639
COHEN, E. B., M. D. GROSSLEIN, and M. P. SISSENWINE. 1982. Energy budget of Georges Bank. Can. Spec. Publ. Fish. Aquat. Sci., 59: 95–107.
DAME, R. F. Jr. 1996. Ecology of marine bivalves, and ecosystem approach. CRC Press, Boca Raton, FL, 254 p.
DAMES, J. K., and R. R. CHRISTIAN. 2006. Uncertainty and the use of network analysis for ecosystem-based fishery management. Fisheries, 31: 331–341. doi:10.1577/1548-8446(2006)31[331:UATUON]2.0.CO;2
EDWARDS, R. L. 1968. Fishery resources of the North Atlantic area. In: The future of the fishing industry of the United States. G. De Witt, (ed.). University of Washington, Seattle, Washington. Publications in Fisheries, 4: 52–60.
EGGERS, D. M. 1977. Factors in interpreting data obtained by diel sampling of fish stomachs. J. Fish. Res. Board Can., 34: 290–294.
FOGARTY, M. J., and S. A. MURAWSKI. 1998. Large-scale disturbance and the structure of marine systems: Fishery impacts on Georges Bank. Ecol. Appl., 8 (1, suppl.): S6–S22. doi:10.2307/2641359
GARCIA, S. M., A. ZERBI, C. ALIAUME, T. DO CHI, and G. LASSERRE. 2003. The ecosystem approach to fisheries: issues, terminology, principles, institutional foundations, implementation and outlook. FAO Tech. Pap., No. 443, 71 p.
GERMAN, A. W. 1987. History of the early fisheries: 1720–1930. Chapter 40. In: Georges Bank. R. H. Backus (ed.). MIT Press, Cambridge, MA, p. 409–424.
GROSSLEIN, M., R. LANGTON, and M. P. SISSENWINE. 1980. Recent fluctuations in pelagic fish stocks of the northwest Atlantic, Georges Bank region, in relation to species interactions. Rapports et Proces-Verbaux Reunions Conseil Int. Explor. Mer, 177: 374–404.
HARLEY, S. J., R. MYERS, N. BARROWMAN, K. BOWEN, and R. ADMIRO. MS 2001. Estimation of research trawl survey catchability for biomass reconstruction of the eastern Scotian Shelf. Can. Sci. Advis. Sec. Res. Doc., 2001/084, 54 p.
HEYMANS, J. J., and D. BAIRD. 2000. Network analysis of the northern Benguela ecosystem by means of NETWRK and ECOPATH. Ecol. Model., 131: 97–119. doi:10.1016/S0304-3800(00)00275-1
HOEY, J. J, E. PRITCHARD, C. BROWN, and M. SHOWELL. 2002. Pelagic shark abundance indices based on fishery dependent and fishery-independent data from the western North Atlantic. Collected Volumes Scientific Papers. ICCAT., 54: 1199–1211.
HUNTLEY, M., and M. LOPEZ. 1992. Temperature dependent production of marine copepods: a global synthesis. Am. Nat., 140: 201–242. doi:10.1086/285410
INNES, S. D., M. LAVIGNE, W. M.EARLE, and K. M. KOVACS. 1987. Feeding rates of seals and whales. J. Anim. Ecol., 56: 115–130. doi:10.2307/4803
JENNINGS, S., M. J. KAISER, and J. D. REYNOLDS. 2001. Marine fisheries ecology. Blackwell Science, Oxford, England. 417 p.
KAVANAGH, P., N. NEWLANDS, V. CHRISTENSEN, and D. PAULY. 2004. Automated parameter optimization for Ecopath ecosystem models. Ecol. Model., 172: 141–149. doi:10.1016/j.ecolmodel.2003.09.004
LARKIN, P. A. 1996. Concepts and issues in marine ecosystem management. Rev. Fish Biol. Fish., 6: 139–164. doi:10.1007/BF00182341
LAURENCE, G. 1979. Larval length weigh relationships for seven species of northwest Atlantic fishes reared in the laboratory. Fish. Bull., 76: 890–895.
LINK, J. S., C. A. GRISWOLD, E. M. METHRATTA, and J. GUNNARD. (eds). 2006. Documentation for the Energy Modeling and Analysis eXercise (EMAX). Northeast Fisheries Science Center Reference Document, 06-15, 166 p.
LINK, J., and J. BRODZIAK. (eds.). 2002. Report on the Status of the NE US Continental Shelf Ecosystem. NEFSC Ecosystem Status Working Group. Northeast Fisheries Science Center Reference Document, 02-11, 245 p.
LINK, J. S. 2002. Ecological considerations in fisheries management: When does it matter? Fisheries, 27: 10–17. doi:10.1577/1548-8446(2002)027<0010:ECIFM>2.0.CO;2
MONACO, M. E., and R. E. ULANOWICZ. 1997. Comparative ecosystem trophic structure of three U.S. mid-Atlantic estuaries. Mar. Ecol. Prog. Ser., 161: 239–254. doi:10.3354/meps161239
MOREL, A., and J. F. BERTHON. 1989. Surface pigments, algal biomass profiles, and potential production of the euphotic layer: relationships reinvestigated in view of remote-sensing applications. Limnol. Oceanogr., 34: 1545–1562.
MURAWSKI S. A., J. J. MAGUIRE, R. K. MAYO, and F. M. SERCHUK. 1997. Groundfish stocks and the fishing industry. In: Northwest Atlantic groundfish: perspectives on a fishery collapse. J. Boreman, B. S. Nakashima, J. A. Wilson and R. L. Kendall (eds.). American Fisheries Society, Bethesda, Maryland, USA, p. 27–70.
NMFS. 1999. Ecosystem-based fishery management. A report to Congress by the Ecosystems Principles Advisory Panel. National Marine Fisheries Service, U.S. Department of Commerce, Silver Spring, MD. 54 p.
O’REILLY, J. E., C. EVANS-ZETLIN, and D. A. BUSCH. 1987. Primary production. Chapter 21. In: Georges Bank. R. H. Backus (ed.). MIT Press, Cambridge, MA, p. 220–233.
O’REILLY, J. E., and C. ZETLIN. 1998. Seasonal, horizontal, and vertical distribution of phytoplankton chlorophyll a in the Northeast U.S. Continental shelf ecosystem. NOAA Tech. Rep. NMFS, No. 139, 120 p.
OVERHOLTZ, W. J. 2002. The Gulf of Maine-Georges Bank Atlantic herring (Clupea harengus): spatial pattern analysis of the collapse and recovery of a large marine fish complex. Fish. Res., 57: 237–254. doi:10.1016/S0165-7836(01)00359-9
PAGES, F. 1997. The gelatinous zooplankton in the pelagic system of the southern ocean: A review. Annales de l’Institut Oceanographique, 73: 139–158.
PALKA, D. 2005. Aerial surveys in the northwest Atlantic: estimation of g(0). In: Proceedings of the workshop on Estimation of g(0) in line-transect surveys of cetaceans. F. Thomsen, F. Ugarte and P. G. H. Evans. (eds.). ECS Newletter, 44 (Special Issue. April 2005): 12–17.
PEARRE, S. 1980. The copepod width-weight relation and its utility in food chain research. Can. J. Zool., 58: 1884–1891.
PECK, M., L. BUCKLEY, E. CALDARONE, and D. BENGSTON. 2003. Effects of food consumption and temperature on growth rate and biochemical based indicators of growth in early juvenile Atlantic cod (Gadus morhua) and haddock (Melanogrammus aeglifinus). Mar. Ecol. Prog. Ser., 251: 233–243. doi:10.3354/meps251233
POMEROY, L. R. 2001. Caught in the food web: complexity made simple? Sci. Mar. (Barc.), 65 (Suppl. 2): 31–40.
POWERS, K. D. 1983. Pelagic distributions of marine birds off the Northeastern United States. U.S. Department of Commerce. NOAA Technical Memorandum, NMFS-F/NEC-27, 201 p.
POWERS, K. D., and E. H. BACHUS. 1987. Energy transfer to birds. In: Georges Bank. R. H. Backus and D. W. Bourne (eds.). MIT Press, Cambridge, MA, p. 372–375.
REEVE, M., and W. WALTER. 1976. A large scale experiment on the growth and predation potential of ctenophore populations. In: Coelenterate ecology and behavior. G. Mackie (ed.). Plenum Pubcorp., New York, NY, p. 187–199.
REID, R. N., D. J. RADOSH, A. B. FRAME, and S. A. FROMM. 1991. Benthic macrofauna of the New York Bight, 1979–1989. NOAA Tech. Rept., NMFS-103, 50 p.
RILEY, G.A. 1941. Plankton Studies. IV. Georges Bank. Bulletin of the Bingham Oceanographic Collection, 40: 1–73.
SAMEOTO, D. 1971. Life history ecological production and empirical mathematical model of the population of Sagitta elegans in St. Margaret’s Bay Nova Scotia. J. Fish. Res. Board Can., 28: 971–985.
SERCHUK F. M., M. D. GROSSLEIN, R. G. LOUGH, D. G. MOUNTAIN, and L. O’BRIEN. 1994. Fishery and environmental factors affecting trends and fluctuations in the Georges Bank and Gulf of Maine Atlantic cod stocks: an overview. ICES Mar. Sci. Symp., 198: 77–109.
SERGEANT, D. E. 1969. Feeding rates of Cetacea. Fiskeridir. Skr. (Havunders.), 15: 246–258.
SISSENWINE, M. P., E. B. COHEN, and M. D. GROSSLEIN. 1984. Structure of the Georges Bank ecosystem. Rapports et Proces-Verbaux Reunions Conseil Int. Explor. Mer, 183: 243–254.
STEIMLE, F. W. Jr. 1985. Biomass and estimated productivity of the benthic macrofauna in the New York Bight: A stressed coastal area. Estuar. Coast. Shelf Sci., 21: 539–554. doi:10.1016/0272-7714(85)90055-1
1987. Benthic faunal production. In: Georges Bank. R. H. Backus (ed.). MIT Press, Cambridge, MA, p. 310–314.
1990. Benthic macrofauna and habitat monitoring on the continental shelf of the Northeastern United States. 1. Biomass. NOAA Tech. Rept., NMFS-86, 28 p.
STILLWELL, C. E., and N. E. KOHLER. 1982, Food, feeding habits, and estimates of daily ration of the shortfin mako (Isurus oxyrinchus) in the Northwest Atlantic. Can. J. Fish. Aquat. Sci., 39: 407–414.
THEROUX, R. B., and R. I. WIGLEY. 1998. Quantitative composition and distribution of macrobenthic invertebrate fauna of the continental shelf ecosystems of the Northeastern United States. NOAA Tech. Rept., NMFS-140, 240 p.
TOWNSEND, D. W., and N. R. PETTIGREW. 1997. Nutrient limitation of secondary production on Georges Bank. J. Plankton Res., 19: 221–236. doi:10.1093/plankt/19.2.221
ULANOWICZ, R. E. 2004. Quantitative methods for ecological network analysis. Comput. Biol. Chem., 28: 321–339. doi:10.1016/j.compbiolchem.2004.09.001
ULANOWICZ, R. E., and J. J. KAY. 1991. A package for the analysis of ecosystem flow networks. Environ. Software, 6: 131–142.
WALTERS, C. J., V. CHRISTENSEN, and D. PAULY. 1997. Structuring dynamic models of exploited ecosystems from trophic mass-balance assessments. Rev. Fish Biol. Fish., 7: 139–172. doi:10.1023/A:1018479526149
WARING, G. T., R. M. PACE, J. M. QUINTAL, C. P. FAIRFIELD, and K. MAZE-FOLEY. (eds.). 2004. U.S. Atlantic and Gulf of Mexico Marine Mammal Stock Assessments – 2003. NOAA Technical Memorandum, NMFS-NE-182, p. 287.
|